10
Nanomedicine in Melanoma: Current Trends and Future Perspectives
Ayman El-Meghawry El-Kenawy1,2
*
• Carolina Constantin3,4 • Snur M. A. Hassan5 • Alshimaa Mohamed Mostafa6 • Adriana Freitas Neves7 • Thaise Gonçalves de Araújo8 • Monica Neagu3,4,9
1Department of Molecular Biology, Genetic Engineering and Biotechnology Research Institute, University of Sadat City, Sadat, Egypt; 2Department of Pathology, College of Medicine, Taif University, Taif, Saudi Arabia; 3Immunology Department, Victor Babes National Institute of Pathology, Bucharest, Romania; 4Colentina University Hospital, Bucharest, Romania; 5Department of Anatomy and Pathology, College of Veterinary Medicine, Sulaimani University, Sulaymaniyah, Kurdistan-Iraq; 6Department of Dermatology, Faculty of Medicine, Beni-Suef University, Beni-Suef, Egypt; 7Molecular Biology Laboratory, Institute of Biotechnology, Universidade Federal de Goias, Catalao, Brazil; 8Laboratory of Genetics and Biotechnology, Institute of Genetics and Biochemistry, Federal University of Uberlandia, Patos de Minas, Brazil; 9 Faculty of Biology, University of Bucharest, Bucharest, Romania
Abstract: As cutaneous melanoma is a highly aggressive and drug-resistant cancer, there is intense research focusing on developing new, efficient drugs. Nanomedicine focuses on developing different groups of nanomaterials for both diagnosis and therapy, and this combination of specific diagnosis and therapy is called theranostics. Nanomaterials tailored as delivery vehicles can be nanocapsules, nanorods, nanotubes, nanoshells, and nanocages. All these structures protect the intended drug against degradation and enhance its stability. The development and characterization of polymeric nanoparticles, polymeric micelles, liposomes, nanohydrogel, dendrimers, inorganic nanoparticles, and hybrid nanocarriers are among the delivery vehicles that transport different anticancer agents. Functionalization of nanocarriers with specific molecules, such as antibodies, can generate different smart nanodrugs for application in cancer therapy and/or diagnosis. Nanotherapeutic strategies deal with several shortcomings that comprise of tumor characteristics, biological barriers, biocompatibility, and so on. As nanostructures interact with various host biomolecules, comprehensive in vitro cellular models call for evaluation of physicochemical properties, dose, and time of action of nanomaterials, while in vivo assessments would provide valuable data regarding the level of absorption, tissue/organ distribution, and metabolism. The future perspectives in nanotechnology applied to cancer overcomes the translational barrier from the laboratory to the clinical application to potentially improve conventional theranostic techniques.
Keywords: Melanoma; Nanomedicine; Nanotechnology; Theranostic; Treatment
Introduction
Melanoma, the cancer of melanocytes, is the sixth most frequently diagnosed cancer in humans and accounts for 80% of skin cancer–related deaths (1). Morbidity and mortality indices are highly variable worldwide—being rare in nations of Asian and African origin and almost considered epidemic in countries of Caucasian predominance (2, 3). When diagnosed early, as a localized cutaneous tumor, melanoma can be surgically removed with a good prognosis (4). Once melanoma becomes metastatic, it turns into a more aggressive and difficult to treat malignancy (5). Management of metastatic melanoma is challenging if the tumor becomes unresectable or if it recurs shortly after resection (6). In such cases, other conventional treatment options including chemotherapy, radiotherapy, targeted therapy, and photodynamic and immunotherapy have to be combined with surgery (7).
Dacarbazine (DTIC) is the first chemotherapeutic treatment approved by U.S. Food and Drug Administration (FDA) for metastatic melanoma (8). Temozolomide, a DTIC derivative, has the ability to cross the blood–brain barrier and is a first-line therapy for brain metastases (9). Recently, BRAF inhibitors (Vemurafenib, Dabrafenib) and MEK inhibitor (Trametinib) have been approved by the FDA for treating BRAF-mutated melanoma which is nearly found in 50% of cases (2). Immunotherapy is another promising treatment option in metastatic melanoma. Ipilimumab, an anti-cytotoxic T-lymphocyte antigen 4 antibody (CTLA-4), and nivolumab and pembrolizumab, programmed death receptor 1 (PD-1) inhibitors, have been approved for use in the treatment of metastatic melanoma (10, 11). However, despite these recent therapeutic breakthroughs, there are still some drawbacks including undesirable side effects, tumor chemoresistance, or even disease relapse (2, 12). As cutaneous melanoma is a highly aggressive cancer (13), there is intense research focus on developing new, efficient drugs. Taken together, these challenges have led researchers to explore new ways of early diagnosis and investigate novel approaches of drug delivery to reach high efficacy, minimal toxicity, and less failure—advantages that melanoma-related nanotechnology could potentially offer (14, 15).
Nanomedicine for Melanoma Detection and Treatment
Early diagnosis of melanoma is essential to increase patients’ survival rates. The 10-year survival rate for Stage IA is 93%, while patients diagnosed at Stage IV have a 10-year survival rate of 10–15% only (16–18). Moreover, the cost of treating melanoma increases dramatically with later stages of the disease (19–21). In addition to the clinical and histological examination, many new techniques have been utilized to aid early detection of melanoma. These techniques include dermoscopy, total body photography, multispectral digital imaging analysis, and RNA microarray (22, 23). In-depth investigations of the molecular changes of metastatic melanoma have paved the way for more advanced technologies known as molecular diagnostics. They include fluorescent in situ hybridization (FISH), next-generation sequencing (NGS), quantitative reverse transcription-polymerase chain reaction (qRT-PCR), comparative genome hybridization (CGH), and detection of exosomes (24–27). Nanotechnology is one of the promising tools recently used for detection of melanoma with high sensitivity and specificity (28–30).
Nanoparticle quantum dots (QDs), fluorescent nanoparticles characterized by excellent brightness, narrow field of emissions, broad absorption spectrum, and excellent photostability, have been suggested as a useful technique for cancer detection (31–35). Those photophysical properties allowed researchers to conjugate QDs with variable cancer-specific molecules as folic acid or antibodies against specific cancer antigens (36–40). When QDs are conjugated with specific anti-melanoma antibodies (e.g., HMB45, MART-1, and Tyrosinase), melanoma cells can be distinguished from normal melanocytes (41). However, the heavy metal composition of QDs, with its high toxicity and immunogenicity, hinders the wide application of QDs as an imaging modality for cancer (42, 43). Recently, coating QDs with a polyethylene glycol (PEG) have been shown to decrease cytotoxicity (44). Similarly, Cornell dots known as C-Dots are PEG-coated silica-based nanoparticles that are used as probes to guide sentinel lymph node biopsy (SLNB) (45, 46). These FDA-approved nanoparticles are used as PET-optical or optical probes that particularly target RGD peptides attached to alpha 2 beta 3 integrin overexpressed in melanoma cells (47–50). Nanotechnology has been used in medicine for developing nanometer scale materials, ranging from 1 to 100 nm, having therapeutic and diagnostic purposes (51–53). Nanomaterials’ size range matches cellular organelles, other molecules involved in intracellular events, as signaling pathways, and/or molecules involved in cell to cell communication (16, 20). The nanomaterials bio-distribution is dependent on the surface charge, biodegradability, size, their distinct biological properties, and shape (19, 21–25). Nanoparticles (NPs) or nanocapsules are the most common shape for nanomaterials used as drug delivery systems. Moreover, this shape offers protection against degradation, enhances its stability, driving an efficient accumulation at target sites (26). Currently, nanorods, nanotubes, nanoshells, and nanocages are nanomaterials with imaging and cancer therapy applications (26, 27).
Carbon-based nanoparticles are effective in melanoma cells (53). Thus, a single-walled carbon nanotube loaded with DOX-induced melanoma cell death in a dose-dependent manner in vitro and revoked tumor development in a xenograft melanoma model. Gold nanoparticles (GNPs) are known as nontoxic, highly stable, easy to synthesize, and minimally interfering with the biological profile of melanoma tumor cell (54, 55). Being of high atomic number and electron density, GNPs are optimal contrast agents for computed tomography (CT) (56, 57). When labeled with radioisotope indium-111 and conjugated with RGD ligands, GNPs were successfully used as radiotracers in experimental melanoma models (58). Meir et al. have shown in melanoma-bearing mice that labeled GNPs can track tumor-specific T-cells using whole body CT. This approach is a next-generation imaging technique as well as a new tool in immunotherapy (59).
Magnetic nanoparticles (MNPs) were successfully used in MRI (60). In the recent MELAMAG clinical trial, SLNB detection based on MNPs was compared to the standard technique. In this study, MNPs with small iron nanoparticles (named Sienna+ by the developers) were intradermally injected and a hand-held magnetometer was used intraoperatively to detect the accumulation of MNPs. A gamma probe was used as comparator and the results showed the feasibility of the magnetic technique for SLNB detection. The highest identification was proven for inguinal and axillary lymph nodes, while the lowest detection rates were registered for the cervical region. From 129 recruited patients, the study reported 95.3% rate of sentinel node identification using this MNPs-based technique (60).
Another nanoparticle tested for contrast-enhanced MRI lymphography is Gadolinium-loaded nanoparticles (Gd-FVT). Using these NPs, the specificity and sensitivity of MRI lymphography in melanoma-bearing mice could be enhanced (61).
Zhou et al. developed an efficient and noninvasive strategy to detect melanoma metastasis in LN using Gd-embedded iron oxide nanoplates (GdIOP), functionalized with Zwitterionic Dopamine Sulfonate (ZDS) molecules. With T1-T2 dual-modal MRI, GdIOP@ZDS nanoparticles were highly taken up by dendritic cells and macrophages in LN, in contrast to melanoma B16 tumor cells which showed lower uptake. This generated difference represented pseudo-contrast images which can be potentially used for detection of melanoma metastasis in LN (62).
RGD-targeted nanoparticles of iron oxide (NPIO) were previously utilized for MRI of in vivo tumor angiogenesis with variable limitations including long blood half-life and nonspecific extravasation (63). Nevertheless, conjugation of cyclic RGD variant [c (RGDyK)], with enhanced affinity for αvβ3, a specific marker of angiogenesis, to iron oxide microparticles (MPIO) provided a more sensitive molecular MRI approach (64).
Another promising application of nanotechnology is the detection and quantification of circulating tumor cells (CTCs) as a blood-based biomarker “liquid biopsy” (65). Seenivasan et al. immobilized anti-Melanocortin 1 receptor antibodies (MC1R-Abs) on amino-functionalized silica nanoparticles (n-SiNPs)polypyrrole (PPy) nanocomposite thin film and used them as an immune sensor for selective and sensitive detection of melanoma cells (66). A magnetite nanoparticle designed by Sato et al. by conjugating N-propionyl-cyst aminyl phenol with magnetite was used in a B16F1 xenograft mouse model (67). Souza et al. showed that melanoma cells were degraded after the application of an external irregular magnetic field to increase the temperature in the tumor to 43°C. The nanoparticle had a 1.7- to 5.4-fold greater effect compared with magnetite alone (46).
Nano Therapies: Radiotherapy and Chemotherapy
NPs in the context of radiotherapy and chemotherapy are particularly interesting. Radiotherapy and surgery are local treatments, while the main systemic strategy is chemotherapy, especially considering the risk of metastasis (30). Radiotherapy has a limited role in treating melanoma patients and is used selectively. Its success is limited due to radiation resistance in melanoma cells (16, 34). This technique has been improved by engineering, physics, chemistry, and biology to promote innovative technologies that allow real-time imaging and better dose distribution according to disease progress (67).
In general, the radioisotopes used in medicine emit energy that produces DNA cleavage, damage that is induced mainly by ionized atoms and free radicals. The clearance performed by the kidney is dependent on the size of the radioisotopes. Molecules smaller than 5 nm are excreted rapidly and fail in promoting desirable effects due to short circulation time in blood. Immune response, including opsonization, is another way for radioisotopes clearance by mononuclear phagocytes (MPS). In this context, nanocarriers emerge as an alternative for the half-life increment of radioisotopes (67). Glutathione-coated gold nanostructures represents the next generation of radiosensitizers used for gamma-ray irradiation (34, 68). Moreover, PEGylation of NPs produces nanocarriers that prevent opsonization, increasing the half-life of the radioisotopes (69). Carbon nanostructures have also been related as potential nanocarriers used in radiotherapy, displaying particular physicochemical properties (70) as ultralight, conductivity, and high-surface area (71).
POLYMERIC NANOPARTICLES
Polymeric nanoparticles (PNs) are molecules usually organized with tunable size into a dense structure with entangling biodegradable polymers presenting thermodynamic stability in an aqueous solvent (72–75). Recently, FDA approved three PNs, namely, polylactic acid (PLA), poly (lactic-co-glycolic acid) 43 (PLGA), and polycaprolactone (PCL). The hydrophilicity for the encapsulation of hydrophilic drugs is one of the deficiencies for the desired release of the encapsulated agents (32). Copolymers as polyethylene glycol (PEG)ylated have been used to reduce the degradation rate of PN to produce PLA-PEG, PLGA-PEG, improving their biocompatibility and modifying its amphiphilicity. Furthermore, PEG has been described as a strategy to evade immune response (76, 77).
LIPOSOMES AND NIOSOMES
Liposomes can remain in the blood circulation longer, permitting continued drug release with increased precision in tumor-targeting (78–84). They can incorporate nucleic acids and other organic or inorganic molecules into their aqueous lumen (85–89) and can be used for targeted, controlled drug release (90–96).
Thus, in two melanoma xenograft models, phosphatidylethanolamine liposomal cisplatin was proven to have higher cytotoxicity than classic liposomes or free cisplatin, a high concentration of intratumoral drug remaining for 72 h and efficiently delivering 3.6-times more drug compared to the free drug (97–103). Niosomes are biodegradable, biocompatible, nontoxic, and nonimmunogenic having extensive solubility and flexibility. Niosomes have been confirmed to have prolonged circulation, increased drug retention in skin, and enhanced drug spreading when topically applied (104–107). Dwivedi et al. proved that encapsulated artemisone which is a 10-amino-artemisinin derivative with antitumor activity in niosomes exhibited extremely selective cytotoxicity toward the melanoma cells but not to the normal skin cells (108).
NANOHYDROGELS
Nanohydrogels are cross-linked hydrophilic soft polymers organized in a tridimensional network comprising a large fraction of water (28, 32). The nanohydrogels’ cross-linking occurs through hydrophilic–hydrophobic interactions, hydrogen bonds, electrostatic interactions, or covalent bonds. The aqueous environment promotes the swelling of nanohydrogels, a characteristic that is determined by the degree of the cross-linking and external environment. This nanocarrier is promising for multimodality treatment, especially for peptides, proteins, and oligonucleotides, because of their hydrophilicity and efficient cell uptake. The co-delivery of PTX and DOX drugs in nanogels are possible due to the positively charged surface that could load negatively charged proteins (32). Functionalized nanohydrogels siRNA delivery systems that target epidermal growth factor receptor were tested in an ovarian cancer mouse model in a platinum-based therapy (82). Polymersome could be valuable for melanoma treatment owing to its benefits, such as robustness, increased drug loading, constancy, relatively longer in vivo circulation, and the possibility to design it for the delivery of multiple drugs (104). Polymersomes have been used to carry DOX for melanoma therapy and established to be specially taken up by melanoma cells (109–111).
THERANOSTIC NANOMEDICINE AND MELANOMA THERAPY
By using nanoparticles for both diagnosis and treatment, theranostic nanomedicine has been advanced recently (112, 113). Liposomes, exosomes, polymersomes, nanocrystals, nanotubes, and nanowires are among the commonly used nanoparticles and nanodevices, and endless combinations can be created with these nanostructures (114). Some metals, such as gold (Au) and Gadolinium (Gd), can have antitumor activity besides being an imaging tracer (88). Gd-based NPs (AGuIX) were successfully used as both MRI contrast agent and therapy in experimental animal models of melanoma metastases (115–120). Another novel theranostic nanostructure for melanoma was a NP biodegradable photoluminescent polymer—poly (lactic acid) (BPLP-PLA) loaded with anti-BRAF V600E– specific drug (PLX4032) and muramyl peptide. The new immune-cell-mediated nanoparticle offers high hopes for melanoma imaging and treatment (121–126).
Current Limitations and Exploring Possibilities for Improving the Efficiency of Nanodrugs in Melanoma
Although notable progress has been made in the synthesis and characterization of nanodrugs, and we are witnessing the first clinical trials that have shown promise (127–130), there are still limitations that should be overcome. Thus, nanodrugs, once having entered the biological system, complexly interact with the host’s immune system, leading to premature clearance, side-effect activation, and toxicity (131–135). Consequently, the main limitations of nanodrug efficacy are the immunological interactions, the biological barriers that hinder the availability of nanodrugs to the intended target, and the heterogeneity of the biological target (38, 136).
In order to improve their efficacy, nanodrugs should overcome these major limitations and several means of overcoming them are further described in this subsection. An overview of the main issues discussed in this chapter is presented in Figure 1.
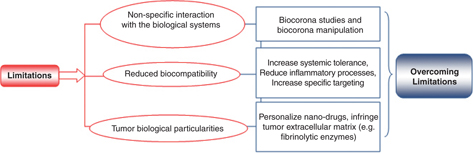
Figure 1 Main limitations of nanodrug efficiency in antitumoral therapy and possibilities to overcome the limitations.
NANODRUGS’ ACTION IS LIMITED BY THE INTERACTION WITH THE BIOLOGICAL SYSTEM
There are complex interactions of nanodrugs once introduced in a biological system, because they would interact with cellular and humoral constituents of the immune system. Thus, the transition to routine clinical application of these nanocompounds would be hampered first by different biological barriers and second by their uncertain fate at the diseased site (136, 137).
In the biological system, nanomaterials interact with all the encountered biomolecules and dynamically form the so called “bio-corona.” The commonly agreed definition of the bio-corona is the multitude and the variety of biomolecules (e.g., proteins, peptides, and lipids) that associate with the surface of a nanoparticle when introduced in a biological system. The process of entrapping nanoparticles within complex surface biomolecules bequeaths them with properties that can hinder the actual intended properties of the nanodrug. Undeniably, the bio-corona establishment controls the nanodrug efficacy and further focuses the actions of natural and adaptive immunity (138). It is not surprising that nanomaterials are directly interacting with the immune system. The evolvement of human immune system was accomplished through exposure to different chemical, physical, and biological agents (139). As NPs are in the size range of biological aggressors, interactions with the immune system are more likely to occur. Thus, a major limitation in nanomedicine is the correct evaluation of the fate of the nanodrugs as antitumoral effectors within a biological system (140–146). As nanomaterials match the same size range as biomolecules and cellular structures endows them with the propensity to reach intracellular structures previously accessible only to biological aggressors. Alternatively, as they have already encountered the complex biological milieu and interacted with other biomolecules, they are subjected to intracellular pathways that are not the intended targets. Hence in vitro investigation of the bio-corona dynamics should be performed to be assured that within the biological system the nanodrugs will reach their intended cellular target (129).
Taking advantage of phagocytes’ capacity to engulf NPs, recently, a novel drug delivery system was reported using macrophages as both carriers and effector cells upon melanoma cells. Hence, nanoparticles of biodegradable photoluminescent poly (lactic acid) were loaded with a drug specific for anti-BRAF V600E mutant melanoma forming a complex engulfed further by macrophages which would directly bind and kill melanoma tumor cells (147–150).
Conclusion
Novel treatment methods should have several properties. For example, they should be more effective, cheaper, and without any risk to patient life, even if they do not improve patient’s quality of life. To accomplish patient safety, and for good patient compliance, an ideal treatment should be developed with an improved overall treatment efficiency, a very low possible toxicity, and a specific targeted site (91). Nanotechnology-based formulations can provide all of the above, and their efficacy can be further improved when ornamented with targeting moieties, for instance, specific antibodies (92) or targeted delivery payload (93–95). In the last 5 years, there has been an exponential increase in the focus on nanotechnology with regard to melanoma therapy and related diagnosis (96). Nanomedicine is a new area that develops nanotechnology for therapeutic and diagnostic purposes. Nowadays, different groups of nanomaterials have been designed for drug delivery and/or for identifying specific markers. Nanomaterials as delivery vehicles can be nanocapsules, nanorods, nanotubes, nanoshells, and nanocages—structures that protect the drug against degradation, thereby enhancing its stability. The development and characterization of PNs, polymeric micelles, liposomes, nanohydrogel, dendrimers, inorganic nanoparticles, and hybrid nanocarriers are among the delivery vehicles that transport different anticancer agents. Chemical drugs, nucleic acids, proteins, antibodies, and functionalization of nanocarriers with inorganic compounds such as magnetic, graphene oxide, carbon, silica, gold and QDs in a core-shell system can generate smart nanodrugs for application in cancer therapy and/or diagnosis. In therapy for skin melanoma, as well as for other tumors, nanotherapeutic strategies deal with several shortcomings that comprise of tumor characteristics, biological barriers, and biocompatibility. Toxicological profile of nanoparticles should be robustly assessed. When systemically administered, nanostructures interact with various host biomolecules, and may trigger toxicity (151, 152). Therefore, comprehensive in vitro cellular models call for evaluation of physicochemical properties, dose, and time of action of nanomaterials, while in vivo assessments would provide valuable data regarding level of absorption, tissue/organ distribution, and metabolism (153). Although preclinical investigations are essential for assessing the potential health risks of nanostructures, animal models retain significant limitations and the human system may react differently to a certain drug compared to animal models (154). Last but not least, the translation of nanodrugs from preclinical to clinical stage is a major issue still unsettled in melanoma nanomedicine area. The future perspectives in nanotechnology applied to cancer is very promising in improving cancer management.
Acknowledgment: Authors Monica Neagu and Carolina Constantin were partially supported through grants PN-II-PCCA-2013-4–1407 (acronym MELTAG, grant no. 190/2014) and COST Action 16120/2017 EPITRAN. Adriana Fretias Neves and Thaise Araujo would like to thank the National Institute of Science and Technology in Theranostics and Nanobiotechnology.
Conflict of interest: The authors declare no potential conflicts of interest with respect to research, authorship, and/or publication of this article.
Copyright and permission statement: To the best of my/our knowledge, the materials included in this chapter do not violate copyright laws. All original sources have been appropriately acknowledged and/or referenced. Where relevant, appropriate permissions have been obtained from the original copyright holder(s).
References
- Bertolotto C. Melanoma: From melanocyte to genetic alterations and clinical options. Scientifica. 2013;12:1–22. http://dx.doi.org/10.1155/2013/635203
- Bombelli FB, Webster CA, Moncrieff M, Sherwood V. The scope of nanoparticle therapies for future metastatic melanoma treatment. Lancet Oncol. 2014;15(1):22–32.
- Heo JR, Kim NH, Cho J, Choi KC. Current treatments for advanced melanoma and introduction of a promising novel gene therapy for melanoma (Review). Onco Rep. 2016;36(4):1779–86.
- Maverakis E, Cornelius LA, Bowen GM, Phan T, Patel FB, Fitzmaurice S, et al. Metastatic melanoma—A review of current and future treatment options. Acta Dermato Venereolog. 2015;95(5):516–27.
- Younes R, Abrao FC, Gross J. Pulmonary metastasectomy for malignant melanoma: Prognostic factors for long-term survival. Melanoma Res. 2013;23:307–11. http://dx.doi.org/10.1097/CMR.0b013e3283632cbe
- Brys AK, Gowda R, Loriaux DB, Robertson GP, Mosca PJ. Nanotechnology-based strategies for combating toxicity and resistance in melanoma therapy. Biotech Adv. 2016;34(5):565–77.
- Lloyd-Hughes H, Shiatis AE, Pabari A, Mosahebi A, Seifalian A. Current and future nanotechnology applications in the management of melanoma: A review. J Nanomed Nanotechnol 2015;6:334. http://dx.doi.org/10.4172/2157-7439.1000334
- Kim T, Amaria RN, Spencer C, Reuben A, Cooper ZA, Wargo JA. Combining targeted therapy and immune checkpoint inhibitors in the treatment of metastatic melanoma. Cancer Biol Med. 2014;11:237–46.
- Schindler AK, Postow MA. Current options and future directions in the systemic treatment of metastatic melanoma. J Com Sup Oncol. 2014;12:20–6. http://dx.doi.org/10.12788/jcso.0005
- Johnson DB, Peng C, Sosman JA. Nivolumab in melanoma: Latest evidence and clinical potential. Therap Adv Med Oncol. 2015;7:97–106. http://dx.doi.org/10.1177/1758834014567469
- Long GV, Atkinson V, Ascierto PA, Dutriaux C, Maio M, Mortier L, et al. Nivolumab improved survival vs dacarbazine in patients with untreated advanced melanoma. J Transl Med. 2015;2:13. http://dx.doi.org/10.1186/1479-5876-13-S1-O6
- Mundra V, Li W, Mahato RI. Nanoparticle-mediated drug delivery for treating melanoma. Nanomed. 2015;10(16):2613–33.
- Ancuceanu R, Neagu M. Immune based therapy for melanoma. Indian J Med Res. 2016; 143(2):135–44. http://dx.doi.org/10.4103/0971-5916.180197
- Chen J, Shao R, Zhang XD, Chen C. Applications of nanotechnology for melanoma treatment, diagnosis, and theranostics. Intl J Nanomed. 2013:8 2677–88. http://dx.doi.org/10.2147/IJN.S45429
- Guy GP, Ekwueme DU, Tangka FK, Richardson LC. Melanoma treatment costs: A systematic review of the literature, 1990–2011. Am J Prev Med. 2012;43(5):537–45.
- Akhtar MJ, Ahamed M, Alhadlaq HA. Therapeutic targets in the selective killing of cancer cells by nanomaterials. Clin Chim Acta Int J Clin Chem. 2017;469:53–62.
- Doane TL, Burda C. The unique role of nanoparticles in nanomedicine: Imaging, drug delivery and therapy. Chem Soc Rev. 2012;41(7):2885–911. http://dx.doi.org/10.1039/c2cs15260f
- Kim BY, Rutka JT, Chan WC. Nanomedicine. N Eng J Med. 2010;363(25):2434–43. http://dx.doi.org/10.1056/NEJMra0912273
- Kumari B, Hora A, Mallik M. Nanomedicines in cancer research: An overview. LS Int J Life Sci. 2017;6:7. http://dx.doi.org/10.5958/2319-1198.2017.00002.1
- Scheinberg DA, Grimm J, Heller DA, Stater EP, Bradbury M, McDevitt MR. Advances in the clinical translation of nanotechnology. Cur Opin Biotechnol. 2017;46:66–73. http://dx.doi.org/10.1016/j.copbio.2017.01.002
- Adiseshaiah PP, Crist RM, Hook SS, McNeil SE. Nanomedicine strategies to overcome the pathophysiological barriers of pancreatic cancer. Nat Rev Clin Onco. 2016;13(12):750–65. http://dx.doi.org/10.1038/nrclinonc.2016.119
- Fernandez-Fernandez A, Manchanda R, McGoron AJ. Theranostic applications of nanomaterials in cancer: Drug delivery, image-guided therapy, and multifunctional platforms. App Biochem Biotechnol. 2011;165(7–8):1628–51. http://dx.doi.org/10.1007/s12010-011-9383-z
- Janib SM, Moses AS, MacKay JA. Imaging and drug delivery using theranostic nanoparticles. Adv Drug Deliv Rev. 2010;62(11):1052–63. http://dx.doi.org/10.1016/j.addr.2010.08.004
- Lammers T, Kiessling F, Hennink WE, Storm G. Nanotheranostics and image-guided drug delivery: Current concepts and future directions. Mol Pharma. 2010;7(6):1899–912. http://dx.doi.org/10.1021/mp100228v
- Lee DE, Koo H, Sun IC, Ryu JH, Kim K, Kwon IC. Multifunctional nanoparticles for multimodal imaging and theragnosis. Chem Soc Rev. 2012;41(7):2656–72. http://dx.doi.org/10.1039/C2CS15261D
- Sanna V, Pala N, Sechi M. Targeted therapy using nanotechnology: Focus on cancer. Int J Nanomed. 2014;9:467–83.
- Sahandi Zangabad P, Karimi M, Mehdizadeh F, Malekzad H, Ghasemi A, Bahrami S, et al. Nanocaged platforms: Modification, drug delivery and nanotoxicity. Opening synthetic cages to release the tiger. Nanoscale. 2017;9(4):1356–92. http://dx.doi.org/10.1039/C6NR07315H
- Ding C, Tong L, Feng J, Fu J. Recent advances in stimuli-responsive release function drug delivery systems for tumor treatment. Molecules. 2016;21(12):pii: E1715. http://dx.doi.org/10.3390/molecules21121715
- Chimene D, Alge DL, Gaharwar AK. Two-dimensional nanomaterials for biomedical applications: Emerging trends and future prospects. Adv Mater. 2015;27(45):7261–84. http://dx.doi.org/10.1002/adma.201502422
- DeVita VT, Jr., Chu E. A history of cancer chemotherapy. Cancer Res. 2008;68(21):8643–53. http://dx.doi.org/10.1158/0008-5472.CAN-07-6611
- Lacerda L, Bianco A, Prato M, Kostarelos K. Carbon nanotubes as nanomedicines: From toxicology to pharmacology. Adv Drug Del Rev. 2006;58(14):1460–70. http://dx.doi.org/10.1016/j.addr.2006.09.015
- Zhang XY, Zhang PY. Nanotechnology for multimodality treatment of cancer. Oncol Let. 2016;12(6):4883–6.
- Ajorlou E, Khosroushahi AY. Trends on polymer- and lipid-based nanostructures for parenteral drug delivery to tumors. Cancer Chem Pharm. 2017;79(2):251–65. http://dx.doi.org/10.1007/s00280-016-3168-6
- Kunz-Schughart LA, Dubrovska A, Peitzsch C, Ewe A, Aigner A, Schellenburg S, et al. Nanoparticles for radiooncology: Mission, vision, challenges. Biomaterials. 2017;120:155–84. http://dx.doi.org/10.1016/j.biomaterials.2016.12.010
- Poon W, Zhang X, Bekah D, Teodoro JG, Nadeau JL. Targeting B16 tumors in vivo with peptide-conjugated gold nanoparticles. Nanotechnology. 2015;26(28):285101. http://dx.doi.org/10.1088/0957-4484/26/28/285101
- Moghimi SM, Farhangrazi ZS. Nanomedicine and the complement paradigm. Nanomedicine. 2013;9(4):458–60. http://dx.doi.org/10.1016/j.nano.2013.02.011
- Weissig V, Pettinger TK, Murdock N. Nanopharmaceuticals (Part 1): Products on the market. Int J Nanomed. 2014;9:4357–73. http://dx.doi.org/10.2147/IJN.S46900
- Bae YH, Park K. Targeted drug delivery to tumors: Myths, reality and possibility. J Control Release. 2011;153(3):198–205. http://dx.doi.org/10.1016/j.jconrel.2011.06.001
- Balch CM, Gershenwald JE, Soong SJ, Thompson JF, Atkins MB, Byrd DR, et al. Final version of 2009 AJCC melanoma staging and classification. J Clin Oncol. 2009;27(36):6199–206.
- Ahlgrimm-Siess V, Laimer M, Arzberger E, Hofmann-Wellenhof R. New diagnostics for melanoma detection: From artificial intelligence to RNA microarrays. Future Oncol. 2012;8(7):819–27. http://dx.doi.org/10.2217/fon.12.84
- Ferris LK, Harris RJ. New diagnostic aids for melanoma. Dermatol Clin. 2012;30(3):535–45. http://dx.doi.org/10.1016/j.det.2012.04.012
- Cooper C, Sorrell J, Gerami P. Update in molecular diagnostics in melanocytic neoplasms. Adv Anat Pathol. 2012;19(6):410–16. http://dx.doi.org/10.1097/PAP.0b013e318271a5cb
- Joyce CW, Murphy IG, Rafferty M, Ryan D, McDermott EW, Gallagher WM. Tumor profiling using protein biomarker panels in malignant melanoma: Application of tissue microarrays and beyond. Exp Rev Proteomics. 2012;9(4):415–23. http://dx.doi.org/10.1586/epr.12.5
- Leachman SA, Cassidy PB, Chen SC, Curiel C, Geller A, Gareau D, et al. Methods of melanoma detection. Cancer Treat Res. 2016;167:51–105.
- Kim MJ, Lee JY, Nehrbass U, Song R, Choi Y. Detection of melanoma using antibody-conjugated quantum dots in a coculture model for high-throughput screening system. Analyst. 2012;137(6):1440–5. http://dx.doi.org/10.1039/c2an16013g
- Zheng H, Chen G, DeLouise LA, Lou Z. Detection of the cancer marker CD146 expression in melanoma cells with semiconductor quantum dot label. J Biomed Nanotechnol. 2010;6:303–11. http://dx.doi.org/10.1166/jbn.2010.1136
- Morosini V, Bastogne T, Frochot C, Schneider R, François A, Guillemin F et al. Quantum dot–folic acid conjugates as potential photosensitizers in photodynamic therapy of cancer. Photochem Photobiol Sci. 2011;10(5):842–51. http://dx.doi.org/10.1039/c0pp00380h
- Fernandes, B. F. et al. Immunohistochemical expression of melan-A and tyrosinase in uveal melanoma. Journal of carcinogenesis. 2007; 6(6): 100–129.
- Kim MJ, Lee JY, Nehrbass U, Song R, Choi Y. Detection of melanoma using antibody-conjugated quantum dots in a coculture model for high-throughput screening system. Analyst. 2012;137(6):1440–5. http://dx.doi.org/10.1039/c2an16013g
- Rizvi SB, Yildirimer L, Ghaderi S, Ramesh B, Seifalian AM, Keshtgar M. A novel POSS-coated quantum dot for biological application. Int J Nanomed. 2012;7:3915–27.
- Lowe AC, Hunter-Ellul LA, Wilkerson MG. Nanotoxicology. Nasir A, editor. Nanotechnology in dermatology. New York: Springer; 2013. p. 231e51.
- Bradbury MS, Phillips E, Montero PH, Cheal SM, Stambuk H, Durack JC, et al. Clinically-translated silica nanoparticles as dual-modality cancer-targeted probes for image-guided surgery and interventions. Integr Biol. 2013;5(1):74–86. http://dx.doi.org/10.1039/C2IB20174G
- Benezra M, Penate-Medina O, Zanzonico PB, Schaer D, Ow H, Burns A, et al. Multimodal silica nanoparticles are effective cancer-targeted probes in a model of human melanoma. J Clin Invest. 2011;121(7):2768–80.
- Friedman R. Nano dot technology enters clinical trials. J Natl Cancer Inst. 2011;103:1428–9. http://dx.doi.org/10.1093/jnci/djr400
- Jain S, Hirst DG, O’Sullivan JM. Gold nanoparticles as novel agents for cancer therapy. Br J Radiol. 2012;85:101–13. http://dx.doi.org/10.1259/bjr/59448833
- Astolfo A, Schultke E, Menk RH, Kirch RD, Juurlink BHJ, Hall C, et al. In vivo visualization of gold-loaded cells in mice using XRay computed tomography. Nanomed. 2013;9:284–92. http://dx.doi.org/10.1016/j.nano.2012.06.004
- Schultke E, Menk R, Pinzer B, Astolfo A, Stampanoni M, Arfelli F, et al. Single-cell resolution in high-resolution synchrotron X-ray CT imaging with gold nanoparticles. J Synchrotron Radiat. 2014;21:242–50. http://dx.doi.org/10.1107/S1600577513029007
- Kim C, Cho EC, Chen J, Song KH, Au L, Favazza C, et al. In vivo molecular photoacoustic tomography of melanomas targeted by bio-conjugated gold nanocages. ACS Nano. 2010;4(8):4559. http://dx.doi.org/10.1021/nn100736c
- Meir R, Shamalov K, Betzer O, Motiei M, Horovitz-Fried M, Yehuda R, et al. Nanomedicine for cancer immunotherapy: Tracking cancer-specific T-cells in vivo with gold nanoparticles and CT imaging. ACS Nano. 2015;9(6):6363–72.
- Anninga B, White SH, Moncrieff M, Dziewulski P, Geh JL, Klaase J, et al. Magnetic technique for sentinel lymph node biopsy in melanoma: The MELAMAG trial. Ann Surg Oncol. 2016;23(6):2070–8. http://dx.doi.org/10.1245/s10434-016-5113-7
- Partridge SC, Kurland BF, Liu CL, Ho RJ, Ruddell A. Tumour-induced lymph node alterations detected by MRI lymphography using gadolinium nanoparticles. Sci Rep. 2015;5:15641. http://dx.doi.org/10.1038/srep15641
- Zhou Z, Liu H, Chi X, Chen J, Wang L, Sun C, et al. A protein-corona-free T 1–T 2 dual-modal contrast agent for accurate imaging of lymphatic tumor metastasis. ACS App Mater Interf. 2015;7(51):28286–93.
- Zhang C, Jugold M, Woenne EC, Lammers T, Morgenstern B, Mueller MM, et al. Specific targeting of tumor angiogenesis by RGD-conjugated ultrasmall superparamagnetic iron oxide particles using a clinical 1.5-T magnetic resonance scanner. Cancer Res. 2007;67(4):1555–62.
- Melemenidis S, Jefferson A, Ruparelia N, Akhtar AM, Xie J, Allen D, et al. Molecular magnetic resonance imaging of angiogenesis in vivo using polyvalent cyclic RGD-iron oxide microparticle conjugates. Theranostics. 2015;5(5):515. http://dx.doi.org/10.7150/thno.10319
- Huang SK, Hoon DS. Liquid biopsy utility for the surveillance of cutaneous malignant melanoma patients. Mol Oncol. 2016;10(3):450–63. http://dx.doi.org/10.1016/j.molonc.2015.12.008
- Seenivasan R, Maddodi N, Setaluri V, Gunasekaran S. An electrochemical immunosensing method for detecting melanoma cells. Biosens Bioelectron. 2015;68:508–15. http://dx.doi.org/10.1016/j.bios.2015.01.022
- Mi Y, Shao Z, Vang J, Kaidar-Person O, Wang AZ. Application of nanotechnology to cancer radiotherapy. Cancer Nanotechnol. 2016;7(1):11. http://dx.doi.org/10.1186/s12645-016-0024-7
- Zhang XD, Chen J, Luo Z, Wu D, Shen X, Song SS, et al. Enhanced tumor accumulation of sub-2 nm gold nanoclusters for cancer radiation therapy. Adv Health Mater. 2014;3(1):133–41. http://dx.doi.org/10.1002/adhm.201300189
- Maeda H. Toward a full understanding of the EPR effect in primary and metastatic tumors as well as issues related to its heterogeneity. Adv Drug Del Rev. 2015;91:3–6. http://dx.doi.org/10.1016/j.addr.2015.01.002
- Jin W, Wang Q, Wu M, Li Y, Tang G, Ping Y, et al. Lanthanide-integrated supramolecular polymeric nanoassembly with multiple regulation characteristics for multidrug-resistant cancer therapy. Biomater. 2017;129:83–97. http://dx.doi.org/10.1016/j.biomaterials.2017.03.020
- Gabizon AA, Patil Y, La-Beck NM. New insights and evolving role of pegylated liposomal doxorubicin in cancer therapy. Drug Resist Updat. 2016;29:90–106.
- Wong JK, Mohseni R, Hamidieh AA, MacLaren RE, Habib N, Seifalian AM. Will nanotechnology bring new hope for gene delivery? Trends Biotechnol. 2017;35(5):434–51. http://dx.doi.org/10.1016/j.tibtech.2016.12.009
- Bae KH, Chung HJ, Park TG. Nanomaterials for cancer therapy and imaging. Mol Cells. 2011;31(4):295–302. http://dx.doi.org/10.1007/s10059-011-0051-5
- Peer D, Karp JM, Hong S, Farokhzad OC, Margalit R, Langer R. Nanocarriers as an emerging platform for cancer therapy. Nat Nanotechnol. 2007;2(12):751–60. http://dx.doi.org/10.1038/nnano.2007.387
- Petros RA, DeSimone JM. Strategies in the design of nanoparticles for therapeutic applications. Nat Rev Drug Discov. 2010;9(8):615–27. http://dx.doi.org/10.1038/nrd2591
- Dobrovolskaia MA, Aggarwal P, Hall JB, McNeil SE. Preclinical studies to understand nanoparticle interaction with the immune system and its potential effects on nanoparticle biodistribution. Mol Pharm. 2008;5(4):487–95. http://dx.doi.org/10.1021/mp800032f
- Yang Q, Lai SK. Anti-PEG immunity: Emergence, characteristics, and unaddressed questions. Wiley Interdiscip Rev Nanomed Nanobiotechnol. 2015;7(5):655–77. http://dx.doi.org/10.1002/wnan.1339
- Hu C-MJ, Zhang L. Nanoparticle-based combination therapy toward overcoming drug resistance in cancer. Bioch Pharmacol. 2012;83(8):1104–11. http://dx.doi.org/10.1016/j.bcp.2012.01.008
- Allen TM, Cullis PR. Liposomal drug delivery systems: From concept to clinical applications. Adv Drug Del Rev. 2013;65(1):36–48. http://dx.doi.org/10.1016/j.addr.2012.09.037
- Zununi Vahed S, Salehi R, Davaran S, Sharifi S. Liposome-based drug co-delivery systems in cancer cells. Mater Sci Eng C Mat Biol Appl. 2017;71:1327–41. http://dx.doi.org/10.1016/j.msec.2016.11.073
- Guo S, Wang Y, Miao L, Xu Z, Lin C-HM, Huang L. Turning a water and oil insoluble cisplatin derivative into a nanoparticle formulation for cancer therapy. Biomaterials. 2014;35(26):7647–53. http://dx.doi.org/10.1016/j.biomaterials.2014.05.045
- Satpathy M, Mezencev R, Wang L, McDonald JF. Targeted in vivo delivery of EGFR siRNA inhibits ovarian cancer growth and enhances drug sensitivity. Sci Rep. 2016;6:36518. http://dx.doi.org/10.1038/srep36518
- Keles E, Song Y, Du D, Dong WJ, Lin Y. Recent progress in nanomaterials for gene delivery applications. Biomat Sci. 2016;4(9):1291–309. http://dx.doi.org/10.1039/C6BM00441E
- Zhang XY, Zhang PY. Mitochondria targeting nano agents in cancer therapeutics. Onco Lett. 2016;12(6):4887–90. http://dx.doi.org/10.3892/ol.2016.5302
- Hidalgo T, Gimenez-Marques M, Bellido E, Avila J, Asensio MC, Salles F, et al. Chitosan-coated mesoporous MIL-100(Fe) nanoparticles as improved bio-compatible oral nanocarriers. Sci Rep. 2017;7:43099. http://dx.doi.org/10.1038/srep43099
- Florinas S, Liu M, Fleming R, Van Vlerken-Ysla L, Ayriss J, Gilbreth R, et al. A nanoparticle platform to evaluate bioconjugation and receptor-mediated cell uptake using cross-linked polyion complex micelles bearing antibody fragments. Biomacromolecules. 2016;17(5):1818–33.
- Haume K, Rosa S, Grellet S, Smialek MA, Butterworth KT, Solov’yov AV, et al. Gold nanoparticles for cancer radiotherapy: A review. Can Nanotechnol. 2016;7(1):8.
- Quyen Chau ND, Menard-Moyon C, Kostarelos K, Bianco A. Multifunctional carbon nanomaterial hybrids for magnetic manipulation and targeting. Bioch Bioph Res Commun. 2015;468(3):454–62. http://dx.doi.org/10.1016/j.bbrc.2015.06.131
- Ahmad MW, Xu W, Kim SJ, Baeck JS, Chang Y, Bae JE, et al. Potential dual imaging nanoparticle: Gd2O3 nanoparticle. Sci Rep. 2015;5:8549. http://dx.doi.org/10.1038/srep08549
- Zhou Z, Forbes RT, D’Emanuele A. Preparation of core-crosslinked linear-dendritic copolymer micelles with enhanced stability and their application for drug solubilisation. Int J Pharm. 2017;523(1):260–9. http://dx.doi.org/10.1016/j.ijpharm.2017.03.032
- Orthaber K, Pristovnik M, Skok K, Peric´ B, Maver U. Skin cancer and its treatment: Novel treatment approaches with emphasis on nanotechnology. J Nanomat. 2017;2017:1–20.
- Bazak R, Houri M, El Achy S, Kamel S, Refaat T. Cancer active targeting by nanoparticles: A comprehensive review of literature. J Cancer Res Clin Oncol. 2015;141(5):769–84. http://dx.doi.org/10.1007/s00432-014-1767-3
- Sultana S, Khan MR, Kumar M, Kumar S, Ali M. Nanoparticles-mediated drug delivery approaches for cancer targeting: A review. J Drug Targ. 2013;21(2):107–25. http://dx.doi.org/10.3109/1061186X.2012.712130
- Wang H, Lee D-K, Chen K-Y, Chen J-Y, Zhang K, Silva A, et al. Mechanism-independent optimization of combinatorial nanodiamond and unmodified drug delivery using a phenotypically driven platform technology. ACS Nano. 2015;9(3):3332–44. http://dx.doi.org/10.1021/acsnano.5b00638
- Bertrand N, Wu J, Xu X, Kamaly N, Farokhzad OC. Cancer nanotechnology: The impact of passive and active targeting in the era of modern cancer biology. Adv Drug Del Rev. 2014;66:2–25. http://dx.doi.org/10.1016/j.addr.2013.11.009
- Daga M, Dianzani C, Ferrara B, Nardozza V, Cavalli R, Barrera G. Latest news on nanotechnology for melanoma therapy and diagnosis. SM J Nanotechnol Nanomed. 2016;1(1):1002.
- Li J, Wang Y, Liang R, An X, Wang K, Shen G, et al. Recent advances in targeted nanoparticles drug delivery to melanoma. Nanomedicine. 2015;11(3):769–94. http://dx.doi.org/10.1016/j.nano.2014.11.006
- Vinogradov S, Wei X. Cancer stem cells and drug resistance: The potential of nanomedicine. Nanomedicine. 2012;7(4):597–615. http://dx.doi.org/10.2217/nnm.12.22
- Urban C, Urban AS, Charron H, Joshi A. Externally modulated theranostic nanoparticles. Trans Cancer Res. 2013;2(4):292.
- Chidambaram M, Manavalan R, Kathiresan K. Nanotherapeutics to overcome conventional cancer chemotherapy limitations. J Pharmacy Pharmaceut Sci. 2011;14(1):67–77. http://dx.doi.org/10.18433/J30C7D
- Dilnawaz F, Singh A, Mohanty C, Sahoo SK. Dual drug loaded superparamagnetic iron oxide nanoparticles for targeted cancer therapy. Biomaterials. 2010;31(13):3694–706. http://dx.doi.org/10.1016/j.biomaterials.2010.01.057
- Tran MA, Watts RJ, Robertson GP. Use of liposomes as drug delivery vehicles for treatment of melanoma. Pigment Cell Melanoma Res. 2009;22(4):388–99. http://dx.doi.org/10.1111/j.1755-148X.2009.00581.x
- Miele, E., Spinelli, G. P., Miele, E., Tomao, F. & Tomao, S. Albumin-bound formulation of paclitaxel (Abraxane® ABI-007) in the treatment of breast cancer. International journal of nanomedicine. 2009; 4: 99–105.
- Bei D, Meng J, Youan B-BC. Engineering nanomedicines for improved melanoma therapy: Progress and promises. Nanomed. 2010;5(9):1385–99. http://dx.doi.org/10.2217/nnm.10.117
- Schilrreff P, Mundiña-Weilenmann C, Romero EL, Morilla MJ. Selective cytotoxicity of PAMAM G5 core–PAMAM G2. 5 shell tecto-dendrimers on melanoma cells. Int J Nanomed. 2012;7:4121–33.
- Pegoraro C, Cecchin D, Gracia LS, Warren N, Madsen J, Armes SP, et al. Enhanced drug delivery to melanoma cells using PMPC-PDPA polymersomes. Cancer Let. 2013;334(2):328–37. http://dx.doi.org/10.1016/j.canlet.2013.02.007
- Rahimpour Y, Hamishehkar H. Niosomes as carrier in dermal drug delivery: INTECH Open Access Publisher. Tabriz University of Medical Science, Iran; 2012.
- Dwivedi A, Mazumder A, Du Plessis L, Du Preez JL, Haynes RK, Du Plessis J. In vitro anti-cancer effects of artemisone nano-vesicular formulations on melanoma cells. Nanomedicine. 2015;11(8):2041–50. http://dx.doi.org/10.1016/j.nano.2015.07.010
- Chaudhuri P, Soni S, Sengupta S. Single-walled carbon nanotube-conjugated chemotherapy exhibits increased therapeutic index in melanoma. Nanotechnology. 2009;21(2):025102. http://dx.doi.org/10.1088/0957-4484/21/2/025102
- Tomalia DA, Khanna SN. A systematic framework and nanoperiodic concept for unifying nanoscience: Hard/soft nanoelements, superatoms, meta-atoms, new emerging properties, periodic property patterns, and predictive Mendeleev-like nanoperiodic tables. Chem Rev. 2016;116(4):2705–74. http://dx.doi.org/10.1021/acs.chemrev.5b00367
- Rej E, Gaebel T, Boele T, Waddington DE, Reilly DJ. Hyperpolarized nanodiamond with long spin-relaxation times. Nature Com. 2015;6:8459. http://dx.doi.org/10.1038/ncomms9459
- Luk BT, Fang RH, Zhang L. Lipid-and polymer-based nanostructures for cancer theranostics. Theranostics. 2012;2(12):1117–26. http://dx.doi.org/10.7150/thno.4381
- Wang LS, Chuang MC, Ho JA. Nanotheranostics—A review of recent publications. Int J Nanomed. 2012;7:4679–95.
- Fraix A, Kandoth N, Manet I, Cardile V, Graziano AC, Gref R, et al. An engineered nanoplatform for bimodal anticancer phototherapy with dual-color fluorescence detection of sensitizers. Chem Commun. 2013;49(40):4459–61. http://dx.doi.org/10.1039/c3cc40714d
- Kotb S, Detappe A, Lux F, Appaix F, Barbier EL, Tran V-L, et al. Gadolinium-based nanoparticles and radiation therapy for multiple brain melanoma metastases: Proof of concept before phase I trial. Theranostics. 2016;6(3):418. http://dx.doi.org/10.7150/thno.14018
- Xie Z, Su Y, Kim GB, Selvi E, Ma C, Aragon-Sanabria V, et al. Immune Cell-Mediated Biodegradable Theranostic Nanoparticles for Melanoma Targeting and Drug Delivery. Small. 2017;13(10): 157–170.
- Ciruelos E, Jackisch C. Evaluating the role of nab-paclitaxel (Abraxane) in women with aggressive metastatic breast cancer. Expert Rev Anticancer Ther. 2014;14(5):511–21. http://dx.doi.org/10.1586/14737140.2014.883922
- Thakor AS, Gambhir SS. Nanooncology: The future of cancer diagnosis and therapy. CA Cancer J Clin. 2013;63(6):395–418. http://dx.doi.org/10.3322/caac.21199
- Oh Y-K, Park TG. siRNA delivery systems for cancer treatment. Adv Drug Del Rev. 2009;61(10):850–62. http://dx.doi.org/10.1016/j.addr.2009.04.018
- Rigel DS, Russak J, Friedman R. The evolution of melanoma diagnosis: 25 years beyond the ABCDs. CA Cancer J Clin. 2010;60(5):301–16. http://dx.doi.org/10.3322/caac.20074
- Li S-Y, Liu Y, Xu C-F, Shen S, Sun R, Du X-J, et al. Restoring anti-tumor functions of T cells via nanoparticle-mediated immune checkpoint modulation. J Control Release. 2016;231:17–28. http://dx.doi.org/10.1016/j.jconrel.2016.01.044
- Sheng W-Y, Huang L. Cancer immunotherapy and nanomedicine. Pharmaceut Res. 2011;28(2):200–14. http://dx.doi.org/10.1007/s11095-010-0258-8
- Hong E, Usiskin IM, Bergamaschi C, Hanlon DJ, Edelson RL, Justesen S, et al. Configuration-dependent presentation of multivalent IL-15: IL-15Rα enhances the antigen-specific T cell response and anti-tumor immunity. J Biol Chem. 2016;291(17):8931–50. http://dx.doi.org/10.1074/jbc.M115.695304
- Beloor J, Choi CS, Nam HY, Park M, Kim SH, Jackson A, et al. Arginine-engrafted biodegradable polymer for the systemic delivery of therapeutic siRNA. Biomaterials. 2012;33(5):1640–50. http://dx.doi.org/10.1016/j.biomaterials.2011.11.008
- Ott PA, Chang J, Madden K, Kannan R, Muren C, Escano C, et al. Oblimersen in combination with temozolomide and albumin-bound paclitaxel in patients with advanced melanoma: A phase I trial. Cancer Chemother Pharmacol. 2013;71(1):183–91. http://dx.doi.org/10.1007/s00280-012-1995-7
- Chen J, Iverson D. Estrogen in obesity-associated colon cancer: Friend or foe? Protecting postmenopausal women but promoting late-stage colon cancer. Cancer Causes Control. 2012;23(11):1767–73. http://dx.doi.org/10.1007/s10552-012-0066-z
- Inamdar GS, Madhunapantula SV, Robertson GP. Targeting the MAPK pathway in melanoma: Why some approaches succeed and other fail. Bioch Pharmacol. 2010;80(5):624–37. http://dx.doi.org/10.1016/j.bcp.2010.04.029
- Dhomen N, Da Rocha Dias S, Hayward R, Ogilvie L, Hedley D, Delmas V, et al. Inducible expression of V600EBraf using tyrosinase-driven Cre recombinase results in embryonic lethality. Pigment Cell Melanoma Res. 2010;23(1):112–20. http://dx.doi.org/10.1111/j.1755-148X.2009.00662.x
- Yin D, Li Y, Lin H, Guo B, Du Y, Li X, et al. Functional graphene oxide as a plasmid-based Stat3 siRNA carrier inhibits mouse malignant melanoma growth in vivo. Nanotechnology. 2013;24(10):105102. http://dx.doi.org/10.1088/0957-4484/24/10/105102
- Kong L-Y, Gelbard A, Wei J, Reina-Ortiz C, Wang Y, Yang EC, et al. Inhibition of p-STAT3 enhances IFN-α efficacy against metastatic melanoma in a murine model. Clin Cancer Res. 2010;16(9):2550–61. http://dx.doi.org/10.1158/1078-0432.CCR-10-0279
- Baldea I, Filip A. Photodynamic therapy in melanoma–an update. J Physiol Pharmacol. 2012;63(2):109–18.
- Yao H, Ng SS, Huo L-F, Chow BK, Shen Z, Yang M, et al. Effective melanoma immunotherapy with interleukin-2 delivered by a novel polymeric nanoparticle. Mol Cancer Ther. 2011;10(6):1082–92. http://dx.doi.org/10.1158/1535-7163.MCT-10-0717
- Camerin M, Magaraggia M, Soncin M, Jori G, Moreno M, Chambrier I, et al. The in vivo efficacy of phthalocyanine–nanoparticle conjugates for the photodynamic therapy of amelanotic melanoma. Eur J Cancer. 2010;46(10):1910–18. http://dx.doi.org/10.1016/j.ejca.2010.02.037
- Sato M, Yamashita T, Ohkura M, Osai Y, Sato A, Takada T, et al. N-propionyl-cysteaminylphenol-magnetite conjugate (NPrCAP/M) is a nanoparticle for the targeted growth suppression of melanoma cells. J Invest Dermatol. 2009;129(9):2233–41. http://dx.doi.org/10.1038/jid.2009.39
- de Souza FF, Dos Santos MC, Dos Passos DCS, de Oliveira L, Celma E, Guillo LA. Curcumin associated magnetite nanoparticles inhibit in vitro melanoma cell growth. J Nanosci Nanotechnol. 2011;11(9):7603–10. http://dx.doi.org/10.1166/jnn.2011.5124
- Lammers T, Kiessling F, Hennink WE, Storm G. Drug targeting to tumors: Principles, pitfalls and (pre-) clinical progress. J Control Release. 2012;161(2):175–87. http://dx.doi.org/10.1016/j.jconrel.2011.09.063
- Neagu M, Piperigkou Z, Karamanou K, Engin AB, Docea AO, Constantin C, et al. Protein bio-corona: Critical issue in immune nanotoxicology. Arch Toxicol. 2017;91(3):1031–48. http://dx.doi.org/10.1007/s00204-016-1797-5
- Simon AK, Hollander GA, McMichael A. Evolution of the immune system in humans from infancy to old age. Proc Biol Sci. 2015;282(1821):20143085
- Fadeel B, Fornara A, Toprak MS, Bhattacharya K. Keeping it real: The importance of material characterization in nanotoxicology. Biochem Biophys Res Commun. 2015;468(3):498–503. http://dx.doi.org/10.1016/j.bbrc.2015.06.178
- Constantin C, Neagu M. Bio-inspired nanomaterials—A better option for nanomedicine. TTRS. 2017;1(1):3–20.
- Nel AE, Madler L, Velegol D, Xia T, Hoek EM, Somasundaran P, et al. Understanding biophysicochemical interactions at the nano–bio interface. Nat Mater. 2009;8:543–57. http://dx.doi.org/10.1038/nmat2442
- Karim R, Somani S, Al Robaian M, Mullin M, Amor R, McConnell G, et al. Tumor regression after intravenous administration of targeted vesicles entrapping the vitamin E α-tocotrienol. J Control Release. 2017;246:79–87. http://dx.doi.org/10.1016/j.jconrel.2016.12.014
- Evangelopoulos M, Parodi A, Martinez JO, Yazdi IK, Cevenini A, van de Ven AL, et al. Cell source determines the immunological impact of biomimetic nanoparticles. Biomaterials. 2016;82:168–77. http://dx.doi.org/10.1016/j.biomaterials.2015.11.054
- Dong X, Chu D, Wang Z. Leukocyte-mediated delivery of nanotherapeutics in inflammatory and tumor sites. Theranostics. 2017;7(3):751–63. http://dx.doi.org/10.7150/thno.18069
- Engin AB, Neagu M, Golokhvast K, Tsatsakis A. Nanoparticles and endothelium: An update on the toxicological interactions. FARMACIA. 2015;63(6):792–804.
- Li R, Ning Z, Majumdar R, Cui J, Takabe W, Jen N, et al. Ultrafine particles from diesel vehicle emissions at different driving cycles induce differential vascular pro-inflammatory responses: Implication of chemical components and NF-kappaB signaling. Part Fibre Toxicol. 2010;7:6. http://dx.doi.org/10.1186/1743-8977-7-6
- Sarna M, Olchawa M, Zadlo A, Wnuk D, Sarna T. The nanomechanical role of melanin granules in the retinal pigment epithelium. Nanomedicine. 2016;13(3):801–7. http://dx.doi.org/10.1016/j.nano.2016.11.020
- Koury J, Zhong L, Hao J. Targeting signaling pathways in cancer stem cells for cancer treatment. Stem Cells Int. 2017;2017:2925869.
- Kumar D, Gorain M, Kundu G, Kundu GC. Therapeutic implications of cellular and molecular biology of cancer stem cells in Melanoma. Mol Cancer. 2017;16(1):7. http://dx.doi.org/10.1186/s12943-016-0578-3
- Kaneti L, Bronshtein T, Malkah Dayan N, Kovregina I, Letko Khait N, Lupu-Haber Y, et al. Nanoghosts as a novel natural nonviral gene delivery platform safely targeting multiple cancers. Nano Lett. 2016;16(3):1574–82. http://dx.doi.org/10.1021/acs.nanolett.5b04237
- Grabbe S, Haas H, Diken M, Kranz LM, Langguth P, Sahin U. Translating nanoparticulate-personalized cancer vaccines into clinical applications: Case study with RNA-lipoplexes for the treatment of melanoma. Nanomed (Lond). 2016;11(20):2723–34. http://dx.doi.org/10.2217/nnm-2016-0275
- Kirtane AR, Sadhukha T, Kim H, Khanna V, Koniar B, Panyam J. Fibrinolytic enzyme cotherapy improves tumor perfusion and therapeutic efficacy of anticancer nanomed. Cancer Res. 2017;77(6):1465–75. http://dx.doi.org/10.1158/0008-5472.CAN-16-1646
- Zhao J, Castranova V. Toxicology of nanomaterials used in nanomedicines. J Toxicol Environ Health B Crit Rev. 2011;14(8):593–632. http://dx.doi.org/10.1080/10937404.2011.615113
- Satalkar P, Elger BS, Shaw DM. Stakeholder views on participant selection for first-in-human trials in cancer nanomedicine. Curr Oncol. 2016;23(6):e530–7. http://dx.doi.org/10.3747/co.23.3214